Levcons
One of the most striking findings was the substantial increase in lift achieved with the incorporation of LEVCON. In the range of angles of attack [Math Processing Error]� = (20–40)[Math Processing Error]°, the generated lift underwent significant improvement due to the phenomenon known as vortex lift. Lift gains ranged from 8.5% to 10% on average, with a peak gain of 19.4%. The energized vortices induced by these devices effectively improved the airflow over the main delta wing, ensuring that the wing maintained lift even at higher angles of attack. Furthermore, the incorporation of LEVCON played a pivotal role in delaying the stall. At high angles of attack, typically around [Math Processing Error]� = (20–35)[Math Processing Error]°, the aerodynamic performance of the aircraft equipped with LEVCON far surpassed that of the configurations without this device.
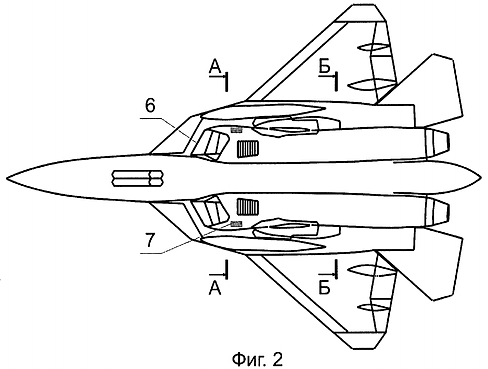
Mission
LEVCONs provide various improvements to delta wing aircraft due to the portion of lift, called vortex lift, that these wings produce. The origins of vortex lift can be traced back to low aspect ratio (AR) wings, which experience complex vortex flows that often lead to separation at the trailing edge. These types of wings exhibit a high angle of attack at maximum lift, a shallow lift curve slope, and reduced longitudinal stability as the angle of attack and sweep angles increase. Increased sweep concentrates the wing load further outboard, potentially causing tip stall. Another crucial aspect is the design of the leading edge, particularly for sweptback wings. Sharp leading edges induce premature flow separation, similar to what occurs with delta wings, even at low angle of attack [17,18].
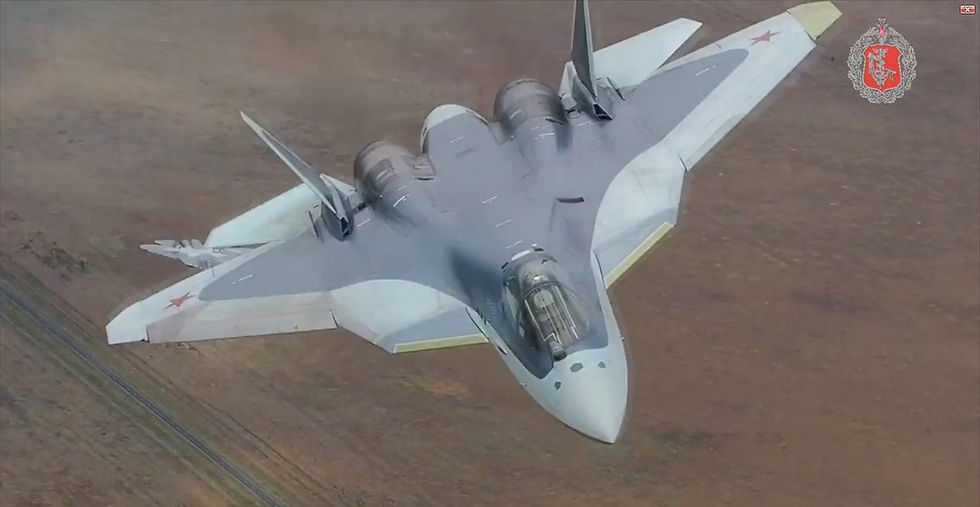
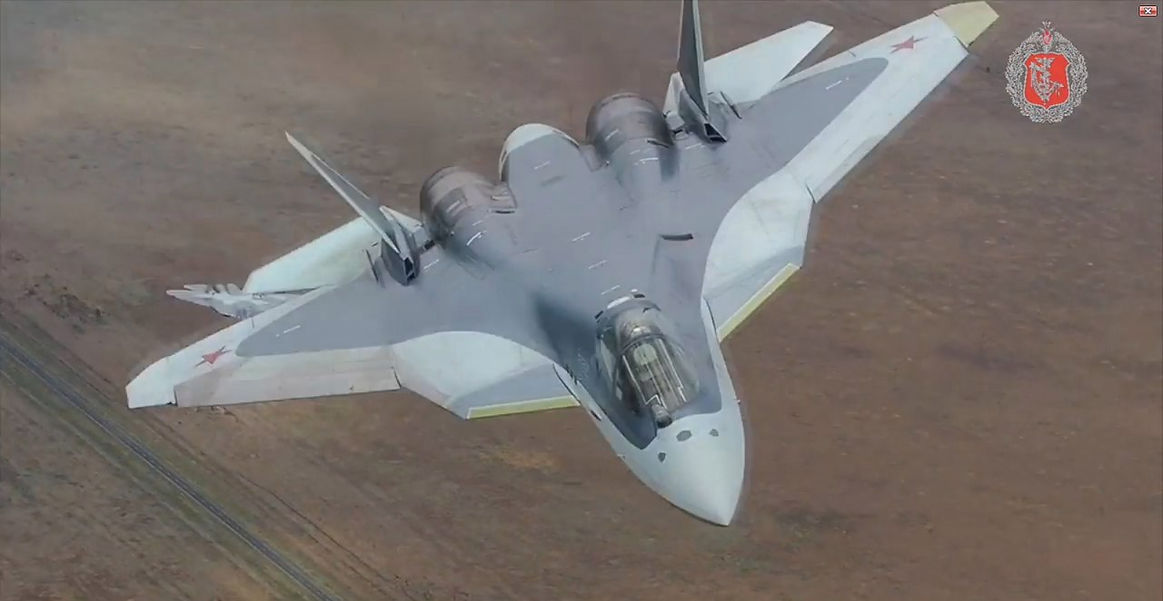
Vision
Experiments conducted on wings with high sweep angles have revealed that the root sections of the wing are not subject to significant peaks in pressure at the leading edge. Additionally, the pressure differences along the spanwise direction cause the boundary layer to move outward from these root sections. These two factors enhance the resistance of the root sections against flow separation, allowing them to generate lift coefficients that exceed the losses experienced when the wing’s tip sections stall. As a result, the wing’s root sections experience stalling at significantly higher angles, whereas the wingtip stalls at much lower angles. As the wing becomes thinner, the angle of attack at which vortex flow becomes observable decreases [17,25]. A major obstacle faced by delta wing aircraft was the significant reduction in the low-speed lift curve slope due to the effects of aspect ratio (AR), which necessitates significantly higher speeds and angles of attack for take-off and landing performance. The discovery of leading-edge vortex flows played a crucial role in resolving this issue [18,26,27]. This breakthrough led to the creation of the first jet-powered delta wing aircraft, the XF-92A. The leading-edge vortex flows discovered in this aircraft enhanced its low-speed, high angle-of-attack flight capabilities. Polhamus [28] summarized various advantages associated with leading-edge vortex flows. Initially, experimentation played a crucial role in the development of aerodynamic configurations, expanding to predict vortex flow aerodynamics. However, existing theories could not predict the intricate details of high angle-of-attack vortex flow aerodynamics. This challenge led to the evolution of theoretical methods, focusing on physics-based modeling of the leading-edge vortices near the lifting wing. These methods began with simplified models and gradually incorporated more intricate physics related to the vortex flows. Eventually, this approach shifted from modeling to capturing the vortices using computational fluid dynamics (CFD) [18].
In summary, vortex lift results from the action of leading-edge vortices and is generated by highly sweptback sharp leading edges or highly swept leading-edge root extensions added to wings with moderate sweep (Figure 2). Vortices generated by the swept wing leading edge are then captured under the airflow and remain trapped over the wing’s upper surface. This results in a negative pressure field over the wing’s top surface. The air flowing over the confined vortex is then pulled downwards, generating lift. Vortex lift generally increases with the angle of attack, but it also has its limits when the vortex bursts. One significant drawback is the increase in the drag component, resulting from the loss of suction behavior around the wing leading edge [29,30]
In addition to enhancing high-alpha capabilities, such as enabling flight at higher angles of attack and reducing stall speed, LEVCONs may also offer an improved lift-to-drag ratio across a broader range of attack angles [15], higher sustained turn rates [14], and enhanced directional controllability [31]. The potential applications of the leading-edge vortex controller are extensive, and it holds promise for providing various benefits to future fighter aircraft [32]. Researchers have already begun incorporating this device into the aircraft models they study [33,34,35]. CFD plays a pivotal role in such studies, given its advantages of lower research costs, time efficiency, and simplification of the prototype phase prior to wind tunnel testing [36,37].
The literature review presented above clearly indicates a lack of comprehensive studies related to vortex lift-augmenting devices that can be implemented in real-life aircraft. Neither vortex flaps nor apex flaps have progressed beyond the research and experimental phases. LEVCONs have already been introduced in two different types of airframes: tail and tailless delta wing combat aircraft. Apart from military aviation, this solution can also be applied to supersonic airliners, given the highly swept delta wings used in this type of aircraft, especially during take-off and landing scenarios.
